The superconductor frenzy, explained.
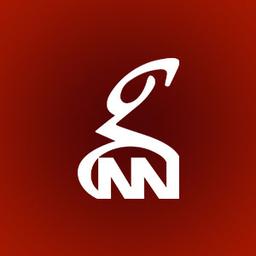
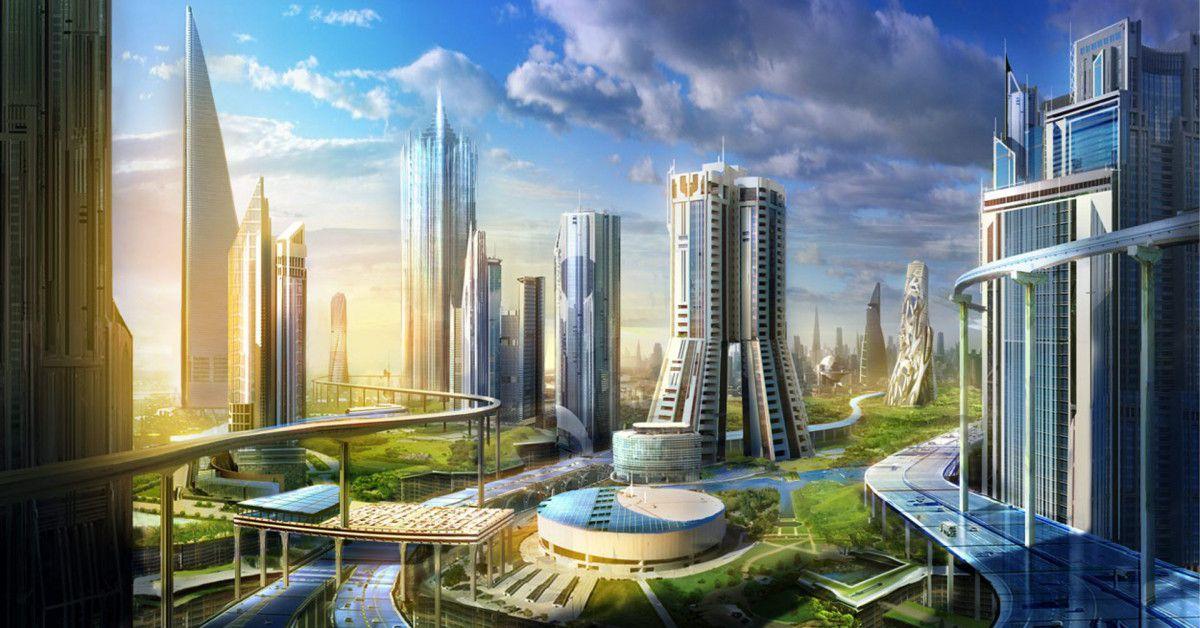
For the past several days, I’ve been frantically reloading Twitter accounts to try to learn as much as possible about LK-99, the purported room-temperature, ambient-pressure superconductor a team of physicists based in South Korea claim to have identified.
This is maybe a week after I learned what a superconductor is, or why it matters that it’s at room temperature or ambient pressure. But within days I went from near-total ignorance to utter glee at the possibilities the technology promises. Provided, of course, it’s real.
You, too, can take this journey from ignorance to giddiness. The details of how to make and investigate superconducting materials are incredibly complex, and the work in question is done by large teams of physicists operating at the cutting edge of the field. But the science of why it matters is, by comparison, relatively simple.
Room-temperature superconducting, if possible, opens the door to staggering technological breakthroughs. It could make transmitting electricity much more efficient; result in faster-charging and higher-capacity electrical batteries; enable practical carbon-free nuclear fusion energy; and make quantum computing — computers capable of solving problems too complex for even the fastest existing computers — feasible at a much larger scale.
A widely useful, easy-to-manufacture superconductor capable of running at normal temperatures would be an enormous breakthrough. Several commentators have compared it to the 1947 invention of the transistor, a technology without which the decades of subsequent progress in computing would not have been possible. Even if LK-99 itself is not that breakthrough, its emergence has revived public interest in superconducting generally, and serves as a useful reminder of how valuable progress in this area could be.
Conductors, and superconductors, explained
Let’s start with the very basics; if you are an electrician or remember more of high school physics than I do, feel free to skip over this part. (Apologies, Mr. Mehrbach, I forgot all this.)
Electricity flows more easily through some materials than others. If a material carries electricity easily, it’s called a conductor; if not, it’s an insulator. Most metals are pretty good conductors, and copper in particular is very good, which is why it’s so often used for electrical wires. (Silver is even better, but much more expensive.) Glass, plastic, and wood are good insulators. I’m using terms like “pretty good” and “very good” because conductivity is a spectrum. Copper doesn’t perfectly transmit electrical charges; it offers some resistance, meaning some electricity loss along the way, just much less than most materials.
At the extreme end of the spectrum are superconductors, which offer literally zero resistance, and perfect conductivity. That such materials exist at all is kind of wild. Normal conductors become more conductive as they cool down, and less as they heat up, but the change is continuous. Superconductors, by contrast, have hard thresholds called “transition temperatures” at which point a material suddenly becomes a superconductor. What’s more, the theory behind why most superconductors work (“BCS theory,” an initialism of the last names of the physicists behind it) is strikingly simple and elegant.
“It’s one of the nicest theories you can have in condensed matter,” Lilia Boeri, a professor of physics at the Sapienza University of Rome and a leading researcher on superconductivity, told me. “It’s a bit like magic. It works beautifully.”
Physicists have known that superconductors exist since 1911, and a number of existing technologies like MRI machines would be impossible without them. But there’s always been a catch. To date, the only known superconductors have to be either extremely cold (lead, for instance, superconducts at negative 447 degrees Fahrenheit) or made of materials that only form at extremely high pressures. (Quite high — a highly controversial recent paper suggested a material that forms at 10,000 times atmospheric pressure, about 10 times the pressure at the bottom of the Pacific Ocean, and skeptics consider that suspiciously low for making a superconductor). Making stuff super-cold and/or applying tons of pressure to it requires considerable energy, which in turns erodes some of the benefit that you get from a superconductor.
In some cases, like MRIs, it’s worthwhile to expend that energy. MRIs require the creation of magnetic fields 30,000 times more powerful than that of the Earth in order to position the nuclei of hydrogen atoms in human bodies so they can be effectively imaged; using liquid helium to cool wires made of a niobium-titanium alloy is cumbersome, but make such a magnet possible. For most purposes outside of MRIs, though, creating a superconductor is just overkill.
A room-temperature, ambient-pressure superconductor would eliminate this trade-off. Creating an incredibly powerful magnet like that used in MRI machines would not require extremely cold temperatures; if this hypothetical superconducting material were easy enough to manufacture, you could create much more powerful MRI machines that use a fraction of the energy used now.
But that would only be the beginning.
A world without lost electricity
Medical imaging is not the only business where people rely on large, powerful magnets. Superconductors are used in some kinds of maglev (magnetic levitation) trains: trains that are not propelled along a track on wheels, but which float above their track and are propelled by magnetic force. With no physical friction from a track, maglev trains can attain extremely high speeds; a working commercial maglev train in Shanghai can hit 268 miles per hour, while a test superconducting maglev system in Japan reached a staggering 373 miles per hour in 2015. Room-temperature superconducting could make systems like this much easier and cheaper to manufacture and operate.
Outside the world of magnets, the potential to transmit electricity with zero loss over long distances, or long time periods, could be even more transformative. Superconductors are already employed in certain limited applications for storing energy. They are used much as a battery might be, but they work through a totally different mechanism. Batteries — from a Duracell AA all the way to a Tesla lithium-ion battery capable of holding approximately 100 kWh — store energy chemically, and can convert it to usable electricity. That necessarily involves some energy loss and inefficiency. Superconducting Magnetic Energy Storage (SMES), by contrast, is just a looping superconductor wire: a circular superconductor that electrons spin around endlessly, never encountering resistance. It’s just an electric current that keeps going and going and going indefinitely, with no loss.
The ability of these systems to instantaneously release a huge amount of power makes them useful as a backup in cases where there’s a sudden loss of power from more ordinary sources. Right now, though, the huge energy required to keep such systems at a low enough temperature that superconducting happens makes their applications limited. But if superconducting could occur at more normal temperatures, SMES systems could be much more widely useful, as their high level of efficiency, durability, and charging/discharging speed compared to batteries could be very attractive, especially for intermittent renewable energy sources that depend on storage.
Superconducting is also important to many nuclear fusion reactor designs. For decades now, physicists have been attempting to generate power by forcing atoms together, the same process that powers the Sun and other stars, with the hope of harnessing a carbon-free energy source but safer and more productive than nuclear fission. But while the creators of the hydrogen bomb were able to do it in an uncontrolled way in 1952, doing it in a controlled way, capable of generating usable electricity, requires containing the reaction in some way. A common theory is to use very powerful magnets, and some designs, like the ITER (formerly the International Thermonuclear Experimental Reactor) in France, rely on superconductors to generate that magnetic force.
Maintaining ultra-low temperatures so superconductors work is one major energy suck of ITER and similar designs, which the reactor needs to overcome to be energy-positive. If it didn’t need to overcome that hurdle, more would be possible. Better superconducting materials, then, or ones requiring much less cooling, could bring us closer to fusion reactors that generate net power.
Then there’s quantum computing. Much like fusion, quantum computing is a long-promised breakthrough that holds the promise to execute certain calculations with much greater speed and precision than ordinary computers are capable of. John Preskill, a leading physicist working on quantum computing, has written that “we have good reason to believe that a quantum computer would be able to efficiently simulate any process that occurs in Nature,” enabling the development of products and systems more effective and efficient than anything that exists today.
Much recent progress in quantum computing has been driven by systems, like Google’s Sycamore processor, that rely on superconductors to work. The need to cool those superconductors to extremely low temperatures has limited the practical usefulness of quantum computers: even adding a wire so that calculations can be transferred from the super-cold superconductors risks heating them up too much. Room-temperature, or more-practical-temperature, superconductors would be a huge help there.
“It’s so over” vs. “we’re so back”
:no_upscale()/cdn.vox-cdn.com/uploads/chorus_asset/file/24835259/LK_99_pellet.png)
So a room-temperature superconductor would be very cool, if real. That leads to the obvious question: Is LK-99, the purported superconductor, real?
The short answer is, I don’t know, and neither does anybody else. Labs around the world, and sometimes individual hobbyists, have been frantically trying to make the material themselves and test to see if it really can superconduct at a high temperature, with mixed results so far. Prediction markets have seen wildly varying odds as participants bet for and against the material working out.
Alex Kaplan, who by day works as head of coffee product for a coffee startup but has a bachelor’s in physics, has recently gained a certain fame as the leader of a Twitter gang tracking LK-99. He narrates the progression of emotions well. First, he was excited. Physicist friends of his were excited, and he fired off a tweet with 30 million views as of this writing proclaiming that this could be “the biggest physics discovery of my lifetime.”
Then disconfirming evidence started to pile up. The physicists in question actually posted two papers (both preprints, yet to undergo peer review): one with three authors— Sukbae Lee, Ji-Hoon Kim, and Young-Wan Kwon (three, incidentally, is the maximum number of people who can share a Nobel Prize in physics), and then one with six. The papers had notable, somewhat suspicious differences. Two coauthors, one listed only on the six-author paper and the second on both, told news agencies that the work was published without their permission. One chart in the six-author paper seemingly showed that the material wasn’t a superconductor, and indeed still had substantial resistance at normal temperatures. Then it emerged that team had authored a separate paper that actually went through peer review and was published in a Korean journal months ago. “The plots were all different” from the later papers, Kaplan notes. “Right away, as soon as I saw, I was like, ‘it’s over.’”
Then it came roaring back — sort of. Sinéad Griffin, a highly respected physicist at Lawrence Berkeley National Laboratory, published a theoretical paper reporting results of a computer model of the material. Griffin wrote the paper in a week, but it was the culmination of work she’s been thinking about for a decade or more. “I had a paper looking at something similar 10 years ago,” she told me. “I knew straight away what was interesting about it.”
The most striking result she found was the little yellow line in the middle of the righthand graph below:
Result 2: The electronic structure of Cu on Pb(1) has isolated flat bands at the Fermi level. These are a direct result of the structural distortion! When I calculate the bands without the distortion, they are not isolated. When I include the distortion, they are! pic.twitter.com/XDgluxPfef
— Sinéad Griffin (@sineatrix) August 2, 2023That flat yellow line is called, appropriately, a “flat band.” Charts like those above are called “spaghetti diagrams,” for obvious reasons, and they act as “a map of what the electrons are allowed to do in your material,” Griffin told me. “Usually in a normal spaghetti diagram, there’s lots of hills and troughs. The atoms are close to each other and interacting with each other.”
If there aren’t hills and troughs, that means that the atoms aren’t interacting much. “It’s weird to see in a material: the atoms are close by, you tend not to have these flat bands,” Griffin continued. “The resulting physics of that is that you have lots of electrons pushed into the same sort of range.” Those electrons then interact with each other. One of the strange results of that interaction can be superconductivity.
Some observers pounced on Griffin’s paper and subsequent modeling studies as evidence that LK-99 really is a superconductor, but Griffin herself is much more cautious. Superconducting is one of the things a structure like this might be able to do, but not the only one: flat bands are sometimes associated with “metal/insulator transition,” in which a material goes from conducting electricity to being an insulator that doesn’t conduct at all. In other words, the exactly opposite of a superconductor.
The next step for her is to use more advanced modeling techniques to nail down what the flat bands and other odd features of the material mean. “The method I used is a good first step, but it has its limitations,” she explains.
In the meantime, physicists and engineers around the world have been trying to make the material themselves. Andrew McCalip, an engineer at commercial space company Varda Space Industries, has been live-tweeting his attempt, culminating in his creation of a rock that floats when placed on a magnet. That behavior, which the original LK-99 paper authors also claimed for their sample, could be evidence of the Meissner effect, which is associated with superconductivity. But it could also be the result of any number of other magnetic reactions.
Researchers at the Huazhong University of Science and Technology reported the same result, as has Iris Alexandra, a pseudonymous Twitter user with an anime avatar who says she’s a soil scientist based in Russia. (Personally I find the Huazhong team most compelling here, but credit must be given to the otaku who got there first.)
It’s really hard to know what to make of these attempts so far. It’s impossible from afar to know if the materials these teams are analyzing are the same as the material the original LK-99 team created, or to independently verify analyses of these materials. More than that, no evidence so far conclusively shows zero resistivity from the material, which is what we’d need to demonstrate to know it’s a superconductor.
“I don’t know why this report attracted so much [attention],” Boeri, the Italian physicist, says. “There are always periodic reports [like this]. This is completely strange, some kind of viral story.”
She worries it could distract from other research efforts in superconductivity, like those involving hydrides: materials combining hydrogen and other elements that, in the superconducting case, so far only form under heavy pressure. One hope is that some materials that can form under such pressure may stay viable, and superconduct, when released to more normal temperatures. “This is something one can imagine taking to scale,” Boeri says. “The materials you make this way are different from materials you have at ambient pressure.”
The claims about LK-99 are extraordinary, and we know what extraordinary claims require. The physicists I spoke with saw no reason to believe it’s a superconductor, based on evidence presented so far. But even if LK-99 fails to replicate as a superconductor, the current hubbub is a good reminder of how useful better high-temperature superconductors could be. Boeri notes that you don’t even need “room temperature” ones for many applications: If a superconductor only needs to be cooled by liquid nitrogen, rather than liquid helium, that’s a huge advantage and energy saver. Existing “high-temperature” superconductors pass that test, but are much too brittle for most practical uses.
Perhaps the best thing to come out of the LK-99 fury is a renewed investment and focus on trying to get more practical superconductors at higher temperatures. The winner might not be LK-99. But there could be a material with similarly magical properties out there yet.
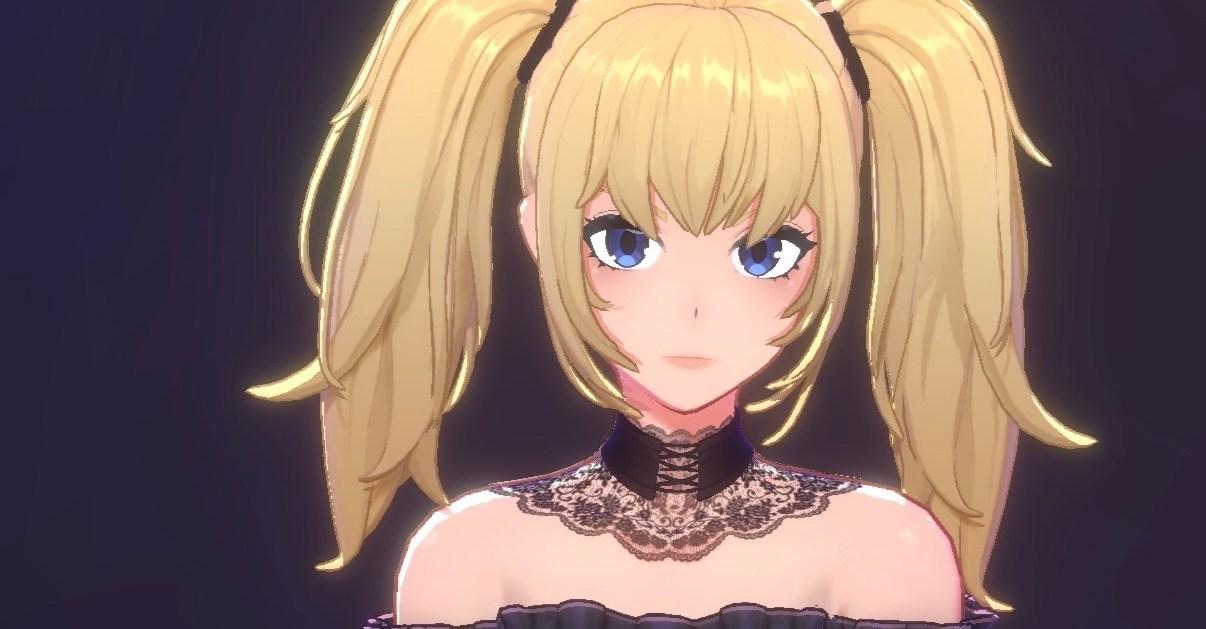
Elon Musk’s AI bot adds a ridiculous anime companion with ‘NSFW’ mode
- 4 گھنٹے قبل
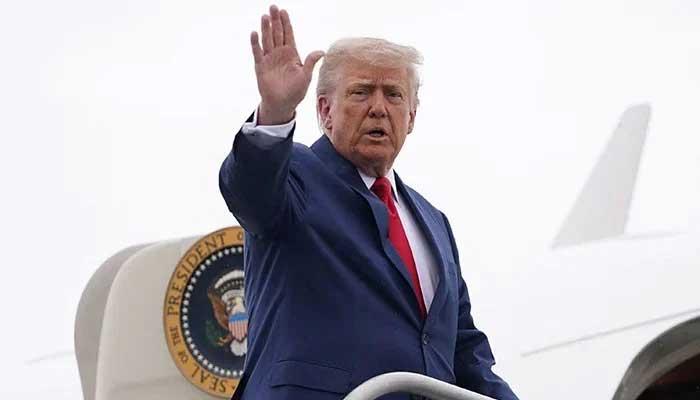
US President Trump likely to visit Pakistan on Sept 18
- 5 گھنٹے قبل
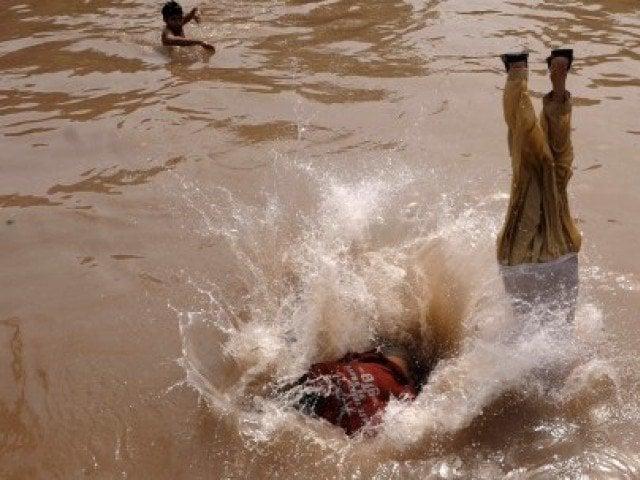
Three children drown while swimming in rainwater in Bahawalnagar
- ایک گھنٹہ قبل
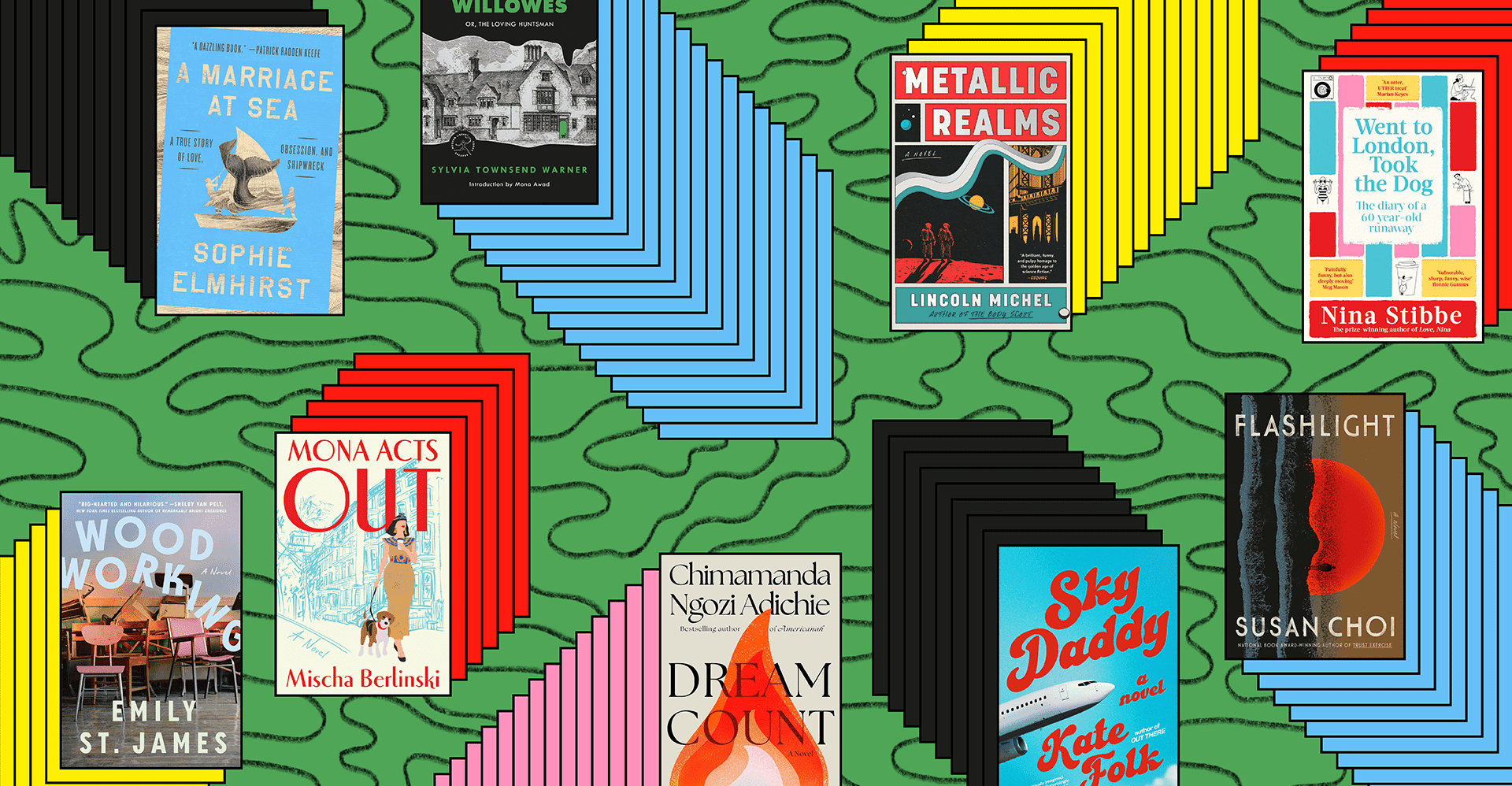
The 9 best books of the year so far
- 2 گھنٹے قبل
Bahrain announces $17bn investment in US across multiple sectors
- 2 گھنٹے قبل
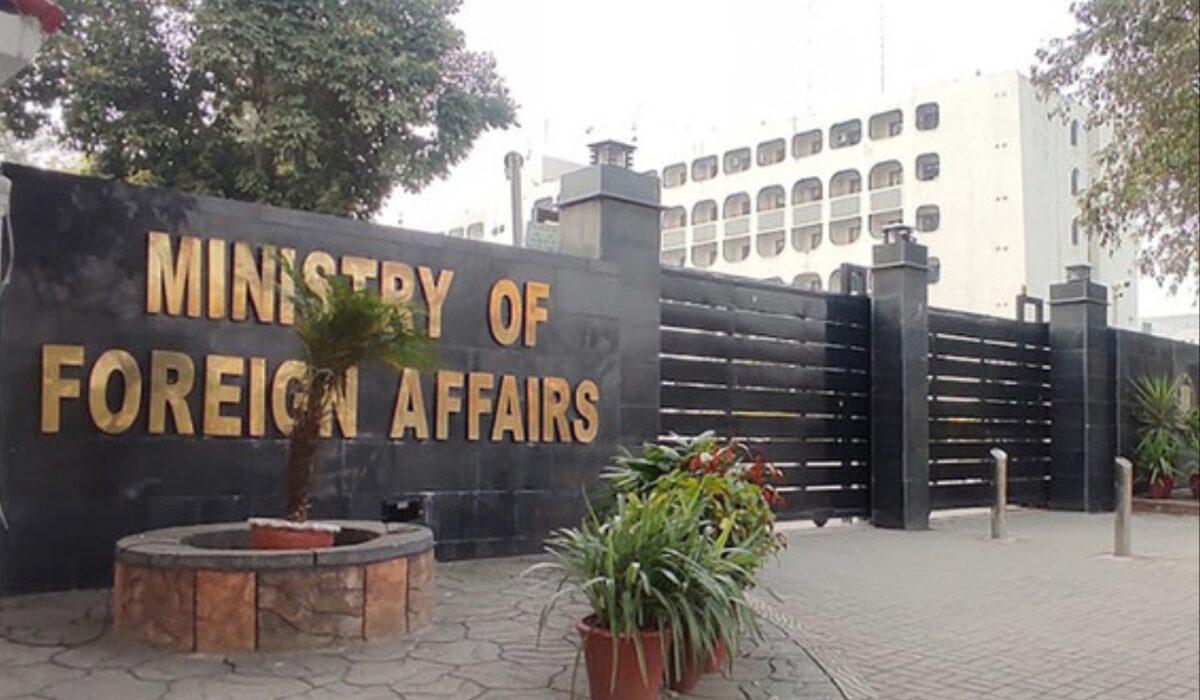
Unaware of US president’s visit to Pakistan, says FO spokesperson
- 2 گھنٹے قبل
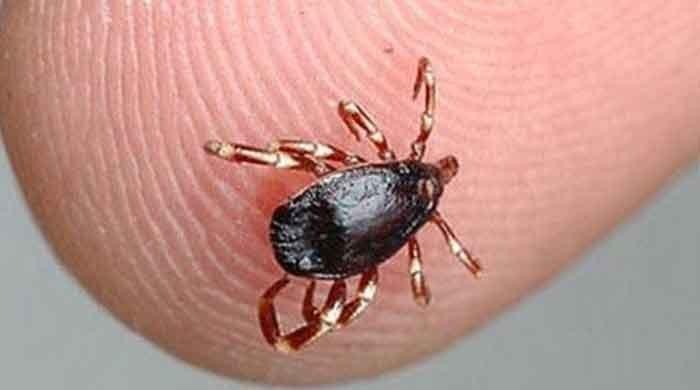
Another Congo virus case emerges in Karachi
- 4 گھنٹے قبل
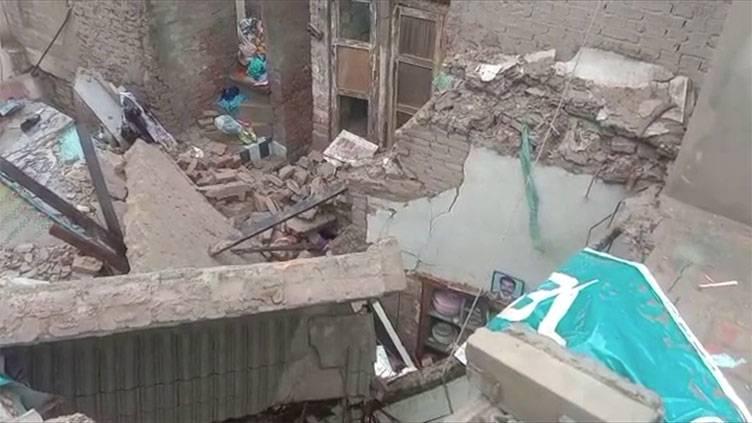
Two women killed in building roof collapse in Karachi
- 2 گھنٹے قبل
Punjab declares emergency after heavy rainstorms kill 33 and injure 170
- ایک گھنٹہ قبل
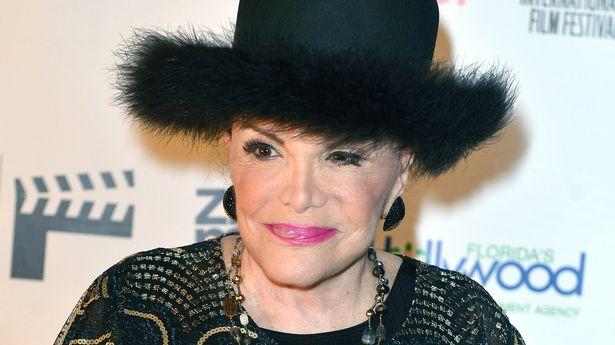
'Pretty Little Baby' singer dies at 87
- 16 منٹ قبل
Israel bombs Syrian army headquarters in latest airstrike
- ایک گھنٹہ قبل
PAF arrives in UK to participate in Royal International Air Tattoo 2025
- 4 گھنٹے قبل